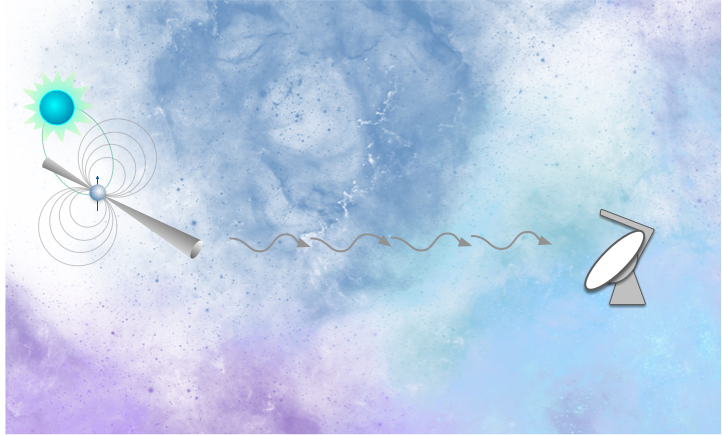
Illustrative diagram describing how a pulsar's signal (arrows) is effected as it travels to our detectors on Earth by not only the Interstellar Medium (background), but also the magnetic field of the pulsar (grew loops) and its orbit around the secondary white dwarf (blue star). Credit: Shami Chatterjee
A Pulsar Timing Array (PTA), such as the one that NANOGrav has constructed, is designed to detect and study long-wavelength gravitational waves. The LIGO detector, which detected gravitational waves from merging black holes in 2015, has similarities and differences with the NANOGrav PTA. In both experiments, however, it is vital to characterize the detector noise that could give rise to a false signal. This is even more important for the NANOGrav PTA because our expected signal is similar to some features of the detector noise. In this paper, we detail how NANOGrav characterizes the detector noise – both its likely causes and its properties – and spell out how we account for that in our search for a gravitational-wave signal in the data.
The NANOGrav PTA consists of 68 arms, each one of which extends from the Earth to a pulsar situated hundreds to thousands of light years distant in our Milky Way Galaxy. As sketched in enclosed Figure, radio waves from each of the 68 pulsars travel a distinct path through the interstellar medium (ISM). Radio waves are slowed down slightly by the ionized gas in the ISM, by an amount that depends on the radio frequency, as is shown by the increasingly delayed signal at lower radio frequencies in Figure 1. The motion of the pulsars through the ISM at hundreds of kilometers per second (and, to a lesser extent, the motion of the Earth and the ISM), causes this dispersion delay to change with time, an effect that we carefully correct for by observing each pulsar at multiple radio frequencies at each epoch. This is but one of more than a dozen such subtle effects that we need to carefully monitor and, where possible, mitigate their effect on the pulse arrival times, which are the essential measurements for our experiment. To achieve the necessary precision, we must predict the arrival of a pulse to within about 1 microsecond over a period of 15 years. This is a fractional precision of 2 parts in 1015, comparable to measuring the distance to the Moon to within a thousandth of a millimeter!
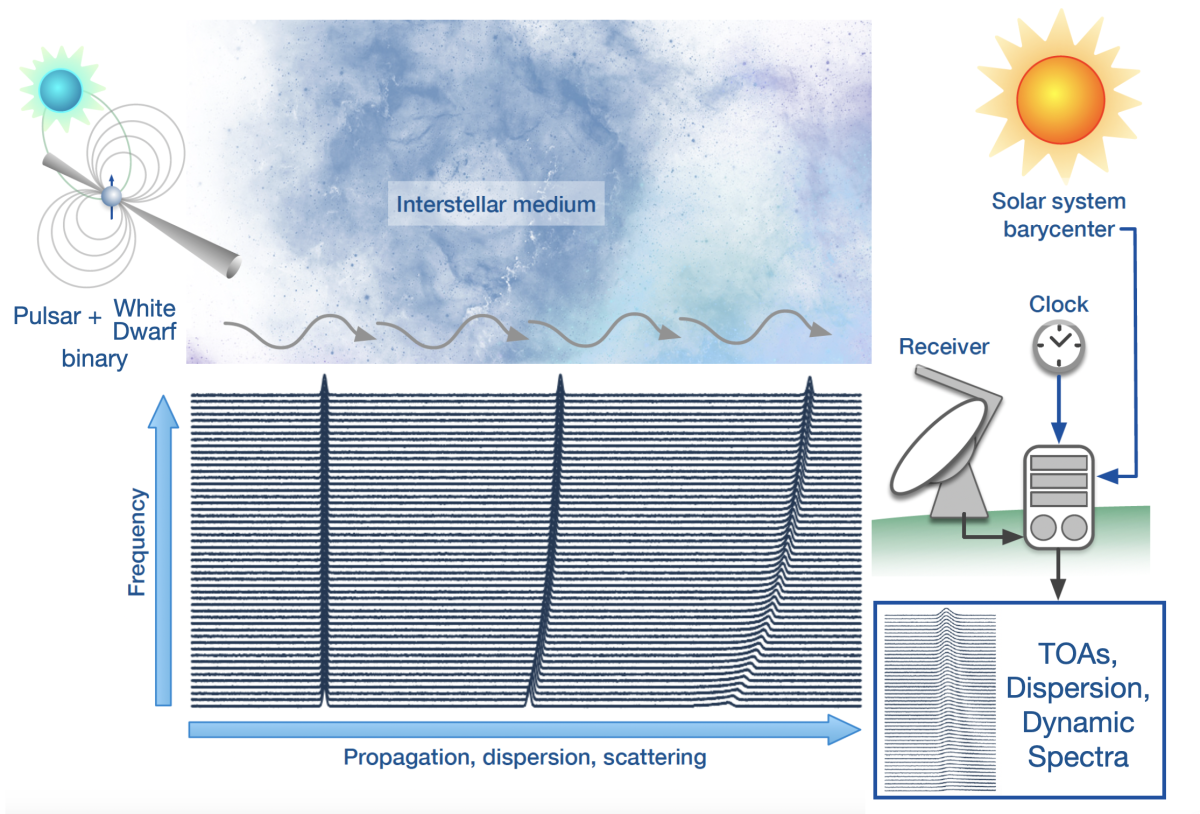
An illustration of the signal path from the pulsar to the data products, highlighting a few relevant sources of noise. The pulsar emission itself is subject to jitter (and in rare cases, glitches), and our model accounts for spin and spindown, the pulsar's sky location, and a binary orbit where needed. Propagation through the interstellar medium imposes pulse dispersion and scattering, which are both frequency-and time-dependent. At the receiver, along with thermal noise, there are effects related to clock corrections and the solar system barycenter, among others, that must be modeled to produce the most accurately measured pulse times of arrival (TOAs) possible. Credit: Shami Chatterjee
Agazie et al., 2023, The NANOGrav 15-Year Data Set: Detector Characterization and Noise Budget. DOI: 10.3847/2041-8213/acda88
Corresponding Author
![]() |
Dr. Jeffrey HazbounLead for the Detector Characterization Paper
|